Untangling the Physics Behind Drifting Embers, ‘Firenadoes’ and Other Wildfire Phenomena
Fires can leap rapidly from building to building and even cause extreme weather events such as pyrocumulonimbus storm clouds
/https://tf-cmsv2-smithsonianmag-media.s3.amazonaws.com/filer/5b/8a/5b8a6df2-b590-4125-830c-a381ba247d63/gettyimages-1018596900.jpg)
Flames begin to rise. Mike Heck jumps back. The tendrils lick upward, wavering in the wind, then coalesce into a vortex of flame, an incandescent tornado writhing in orange and red. “There it goes!” says one onlooker. Another whistles in astonishment.
But nobody is concerned. Heck set the fire deliberately, igniting a pan of liquid on the floor of a room lined with concrete blocks to contain the flames. A suction hood overhead prevents smoke from billowing into nearby classrooms.
Heck’s supervisor, fire scientist Michael Gollner of the University of Maryland in College Park, regularly conjures up such blazing pillars, known as fire whirls, in his lab. (Gollner and colleagues explore the science of these phenomena in the 2018 Annual Review of Fluid Mechanics.) From them, and from other fiery experiments, he aims to learn how flames intensify and spread as cities and landscapes burn. Gollner’s goal is to better understand what drives fire to leap its way from house to house and from tree to tree.
Gathering new insights into fire behavior has become increasingly urgent as wildfires have become more extreme, particularly in western North America. Starting in the mid-1980s, big wildfires suddenly became much more common in western US forests, especially in the northern Rocky Mountains. More recently, forests in the Pacific Northwest have seen the biggest increase in wildfire sizes, with a nearly 5,000 percent increase in burn area from 2003 to 2012 compared with the 1973–1982 average. Nationwide, the average acreage burned in the years since 2000 is nearly double the annual average for the 1990s.
And just in the last two years, several deadly infernos have incinerated parts of California. More than 5,600 buildings burned to the ground in and around Santa Rosa in October 2017. Last July in Redding, a towering plume of hot air and ash spawned a spinning “firenado” like the one in Gollner’s lab—but much bigger, and ferocious enough to kill a firefighter. The same month, fires burned vast acreage in Mendocino and three other counties. Four months later, 85 people died in the Camp Fire in Paradise, many of them incinerated while trying to escape the blaze in their cars.
Record-Breaking Ravages
All told, the state’s recent fires set records for California’s biggest, deadliest and most destructive wildfires. “Nature has given an astonishing sequence of events, each one outdoing the one before,” says Janice Coen, an atmospheric scientist who studies wildland fires at the National Center for Atmospheric Research in Boulder, Colorado. She and others find themselves asking: “Is this different from the past? What’s going on here?”
/https://tf-cmsv2-smithsonianmag-media.s3.amazonaws.com/filer/d4/86/d486b525-65cc-4925-8f7b-1a4382e2ad75/g-wildland-fires-graph_1.jpg)
Many factors have driven this unprecedented expansion of wildfire devastation. Decades of reflexively snuffing out fires as soon as they ignited have allowed fire-fueling shrubs and trees to accumulate in unburned areas. Climate change brings warmer temperatures, less rain and snowpack, and more chances for fuels to dry out and burn. (Human-caused climate change has been blamed for nearly doubling the forest area burned in the western United States since 1984.) Meanwhile, more people are moving into wildland areas, increasing the chance that someone will ignite a fire or be in harm’s way when one begins to grow.
Coen and other scientists are tapping physics to help reveal what causes an ordinary blaze to escalate into an epic megafire. To do this, some researchers drive to the edges of wildfires, probing their secrets with laser and radar equipment that can see through the billowing smoke clouds. Others have developed cutting-edge models that describe how flames race across the landscape, driven not only by fuels and terrain but also by how the fire and atmosphere feed back on one another. And still others, like Gollner, are devising laboratory experiments to figure out why one house may ignite while its neighbor remains unscathed.
Such findings may show how people can better prepare for a future with more intense wildfires, and perhaps how firefighters can more effectively combat them.
Fire Weather
When it comes to battling blazes, “there’s a lot of reliance on what people have seen fires do in the past,” says Neil Lareau, a meteorologist at the University of Nevada, Reno. “That personal deep experience is really valuable, but it breaks down when the atmosphere goes into what I would call outlier mode—when you’re going to be witnessing something you’ve never seen before.”
So Lareau works to gather information about fires as they unfold, hoping to one day be able to deliver specific warnings for firefighters as they battle the flames. He understands the danger more than many academic researchers do: He spent three summers trying to get as close to wildfires as he could, as part of the renowned fire-meteorology research team led by Craig Clements of San Jose State University in California.
Like the storm chasers who stalk tornadoes on the Midwest plains, fire chasers have to be prepared for anything. They go through firefighter training, learning how to anticipate where the fire line might move and how to deploy a fire shelter in an emergency. They register with the federal emergency management system so they can be officially invited into areas where the public can’t go. And they travel with a sophisticated laser-scanning machine in the back of one of their trucks for penetrating the ash and smoke plumes rising off an active fire.
“Just by virtue of pointing our laser at things, we started seeing things people had not documented in the past,” Lareau says. Early discoveries include why a fire’s plume spreads out as it rises while smoky air is pushed outward and clear air is folded inward, and how rotating columns of air can form within the plume. “There’s this fascinating environment where fire and atmospheric processes interact with one another,” he says.
/https://tf-cmsv2-smithsonianmag-media.s3.amazonaws.com/filer/9b/e7/9be74a1a-e417-4d0e-a66e-525460520f16/g-how-pyrocumulonimbus-forms_0.jpg)
One of the most dramatic examples of “fire weather” is the thunderstorm-like clouds that can appear high above a fire. Called pyrocumulonimbus clouds, they form when there is relatively high humidity in the atmosphere. A plume of ash and hot air rises rapidly from the fire, expanding and cooling as it gets higher. At some point, typically about 15,000 feet high, it cools off enough that water vapor within the air condenses into a cloud. The condensation releases more heat into the plume, reinvigorating it and generating a bright white cloud that can tower up to 40,000 feet high.
Beneath the cloud base, air can rush upward at speeds approaching 130 miles an hour, driven by convection within the plume, the San Jose State team has discovered. The more the fire grows, the more air gets pulled into the updraft, intensifying the entire conflagration. And in rare cases it can even spawn a flaming tornado below.
Birth of a Fiery Tornado
Lareau watched a firenado form almost in real time during the Carr fire, near Redding, in July 2018. In this case he wasn’t nearby with a laser in his truck, but sitting at a computer looking at radar data. Weather radars, like those used for your local forecast, can track the speed of small particles such as ash moving in the air. As the Carr fire developed, Lareau pulled up radar data from a military base nearly 90 miles from the growing fire. By watching how the ash moved in opposite directions at different levels in the atmosphere, he could see how atmospheric rotation within the plume was shrinking and intensifying. Like figure skaters pulling their arms in during a spin, the rotation contracted and sped up to form a coherent vortex—a tornado embedded in the larger ash plume.
It is only the second known example, after a 2003 firestorm in Australia, of a tornado forming because of a pyrocumulonimbus cloud, Lareau and colleagues wrote in December in Geophysical Research Letters. The fire provides the initial heat that generates the cloud, which then generates the tornado. “The dynamics that lead to the rotation collapse aren’t just driven by fire, they are also driven by the cloud itself,” Lareau says. “That’s really what’s different about this case, compared to your more garden-variety fire whirl.”
Imagine a twister in the midst of a conflagration, and it’s easy to see why the Carr fire was so devastating. With wind speeds topping 140 miles an hour, the fire tornado knocked down electrical towers, wrapped a steel pipe around a power pole and killed four people.
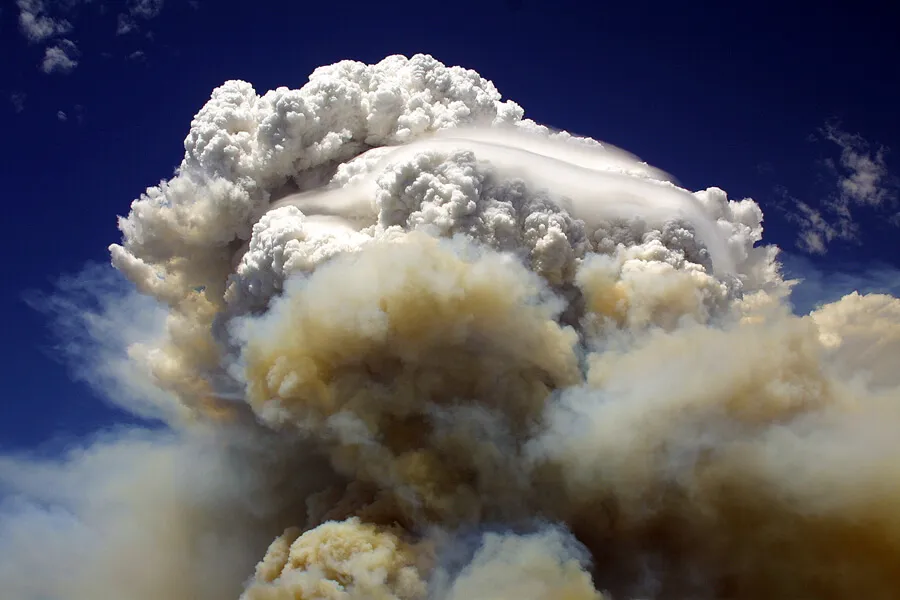
Predicting Flames’ Next Move
That sort of devastation is what drives Coen to model wildfires. She grew up just outside of Pittsburgh, the daughter of a firefighter, and later became entranced by how winds, eddies and other atmospheric circulation help drive the spread of flames. Depending on how the air flows across the landscape, a fire can shift where it is moving—perhaps splitting into two parts and then merging again, or popping off little eddies or whirls along the fire line. “Foresters think about fires as fuel and terrain,” Coen says. “To us, as meteorologists, we see a lot of phenomena we recognize.”
In the 1980s and 1990s, meteorologists began to link weather models, which describe how air flows over complex terrain, with those that predict fire behavior. One such system, a computer model developed at the US Forest Service’s Missoula Fire Sciences Laboratory in Montana, is now regularly used by federal agencies to forecast where fires will grow.
Coen went a step further and developed a joint atmosphere-and-fire model that incorporates airflow. It can, for instance, better simulate how winds eddy and break around peaks in steep terrain.
Her model became shockingly real on November 8, 2018, when she was scheduled to give a talk, “Understanding and Predicting Wildfires,” at Stanford University. The night before, while working on her presentation, she saw reports that the Pacific Gas and Electric Company was considering shutting down equipment in parts of the Sierra Nevada foothills because strong winds were forecast.
The next morning she went to the symposium but sat in the back searching the Internet and listening to emergency radio feeds. As colleagues spoke, she followed scanner traffic, hearing that a fire had ignited in Northern California and spread quickly toward the town of Paradise. “That’s when I had to launch into my presentation,” she says. “I could tell by the winds, by how badly the evacuation was going, that it was going to be a horrible event. But at that point we didn’t know it would be the most deadly one in California history.”
Those strong winds she had heard about turned out to be crucial to how the fire spread and engulfed Paradise. Strong downslope winds pushed the flames into the heavily forested town. It was entirely predictable according to the physics in her models, Coen says: “A lot of weird things make sense after you look at these fine-scale circulations.”
Another example is the Tubbs fire that devastated Santa Rosa in October 2017, roaring across 12 miles in just over three hours. Coen’s models explore how airflows known as the Diablo winds move across the landscape. It turns out that a layer of stable air slid quickly over the complex topography above Santa Rosa. Where it hit mountain ridges, it generated bursts of high-speed winds. Surprisingly, the wind bursts didn’t come off the highest peaks, but rather a smaller set of peaks that were downwind. The location of some of those wind bursts, which reached up to 90 miles an hour according to her model, corresponds to where the fire ignited—perhaps because of electrical equipment failures. Coen described the work in Washington, DC, in December at a meeting of the American Geophysical Union.
Coen’s models also help explain the Redwood Valley fire, which started in the same windstorm as the Tubbs fire. (Fourteen separate fires broke out in Northern California in the span of 48 hours, as a high-pressure weather system inland sent Diablo winds rushing offshore.) But in this case there was a seven-mile-wide gap in the mountains that winds were able to rush through, compressing and speeding up. It was like a single narrow river of winds—which would be hard to spot with traditional weather or fire forecasts, Coen says. “If you were looking at the weather data and saw this one situation was unusual compared to the rest, your mind would tend to dismiss it,” she says.
But forecasters need to pay attention to those blips of high-speed wind readings. They could be signaling that something very localized—and very dangerous—is going on.
From Spark to Combustion
Researchers like Coen track the spread of a fire’s perimeter to predict where the active fire line might move. But physics can also help scientists better understand another type of fire spread: what happens when the winds catch embers and loft them miles ahead of the fire front. When they land, those embers can sometimes smolder in place for hours before igniting a pile of leaves, a deck or something else flammable. That’s a big problem for firefighters trying to figure out where to deploy their resources—whether to stay on the main fire line or to chase where they think spot fires might ignite.
To get at this question, back at the University of Maryland Gollner has been working out the small-scale physics of what it takes for an ember to ignite. His laboratory is in the Department of Fire Protection Engineering, and it looks the part. Butane lighters fill drawers. A box of pine straw rests on a shelf. Thick fire-protective gloves lie atop a stool. The air smells mildly acrid, like the whiff of a fire just extinguished.
Along one wall of the lab, beneath a large ventilation hood, Gollner shows off a metal contraption a little flatter and wider than a shoebox. This is where he creates an ember by igniting a cork-shaped piece of wood and putting it inside the box. A fan blows a constant breeze over the smoldering firebrand, while instruments beneath the box measure the temperature and heat flow of the surface it is sitting on. With this device Gollner can study what it takes for embers to generate enough heat to start a building fire. “A lot of studies have been done on beds of grasses and fine stuff,” he says. “We wanted to understand, how does it ignite your deck, your roof or your structure?”
It turns out that a single ember, or a handful of embers, can’t build up that much heat if it lands on a material such as a deck or a roof. But put one or two dozen embers into Gollner’s device and the heat flux goes up dramatically, he and his colleagues report in the March Fire Safety Journal. “You start to have re-radiation between them,” he says. “It glows, under the wind—it’s just beautiful.”
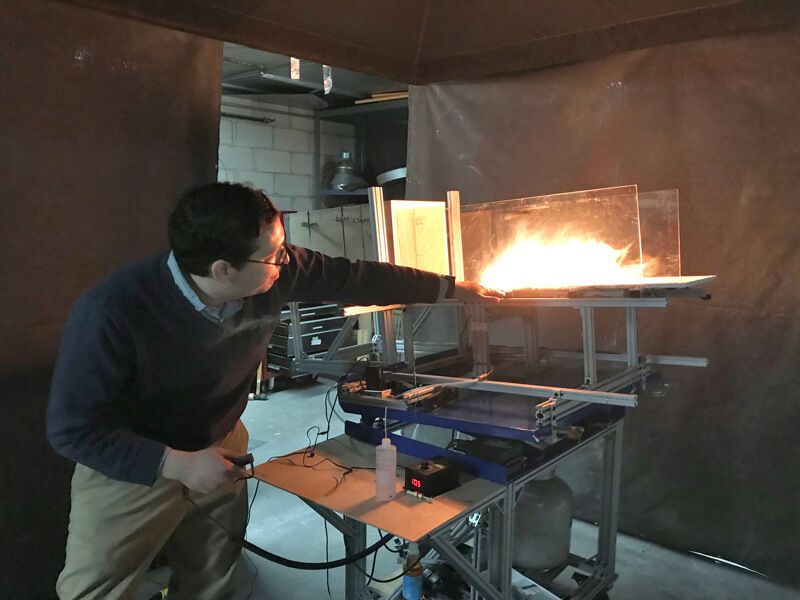
Just a small pile of embers can generate about 40 times the heat you’d feel from the sun on a hot day. That’s as much heating, and sometimes more, as comes from the fire itself. It’s also enough to ignite most materials, such as the wood of a deck.
So if there are a lot of embers flying ahead of a fire, but those embers land relatively far from one another, they may not build up the radiative heat needed to generate a spot fire. But if the embers pile up, perhaps blown by the wind into a crevice of a deck, they can smolder together and then trigger an ignition, Gollner says. Most homes that burn in the wildland-urban interface ignite from these embers, often hours after the fire front itself has passed.
Understanding the heat flux at these small scales can illuminate why some houses burn while others don’t. During the Tubbs fire, homes on one side of some streets were destroyed while those on the other side had hardly any damage. That may be because the first house that ignited radiated energy to its neighbor, which then burned neighboring homes like dominoes because of the radiative heat. When houses are closely packed together, there’s only so much homeowners can do to mitigate the danger by clearing brush and flammable material around the house.
Controlling the Beast
Gollner—a California native who grew up evacuating from wildfires—is now working on other aspects of fire spread, like what it takes for a flaming piece of vegetation to break off in high winds and ignite other shrubs downwind. He is studying fire whirls to see if they can be used to burn off oil slicks in the ocean, since whirls burn the oil faster and more cleanly than a nonrotating fire. And he is beginning a project on the health effects of inhaling wildfire smoke.
For now, he hopes his research can help save homes and lives during an active fire. “You’re never going to make anything fireproof,” he says. “But as you make it better you make a big difference.” Homes built with shields against embers coming in through attic openings, or using ignition-resistant materials like asphalt instead of wood shingles, may be less likely to ignite than homes not built to those standards. If only 10 homes and not 1,000 ignite during a firestorm, firefighters might be able to better manage the next big conflagration, Gollner says.
As climate warms and fires grow more extreme, fire scientists know their work is more relevant than ever. They are pushing to make their research matter where it counts—on the front lines with emergency management officials. Coen, for instance, is working to run her wildfire models faster than real time, so that when the next big fire breaks out she can quickly predict where it might go given the wind and other atmospheric conditions. And Lareau is developing ways to track a fire’s spread in near real time.
He uses weather information like the ground-based radar he used to track the Carr firenado, as well as satellites that can map the fire perimeter by studying heat flowing off the ground. Eventually, he wants to see a real-time prediction system for wildfires like those that currently exist for thunderstorms, tornadoes, hurricanes and other weather events.
“The warnings aren’t going to stop the fire,” says Lareau. “But maybe it will help us decide where to make those decisions. These are environments where minutes matter.”
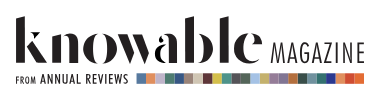
Alexandra Witze (@alexwitze) is a science journalist living in the wildland-urban interface above Boulder, Colorado, where she occasionally sees smoke from nearby fires.