The Mars Dilemma
When astronauts finally reach Mars, will they be able to land?
/https://tf-cmsv2-smithsonianmag-media.s3.amazonaws.com/filer/b8/85/b8855ec1-9695-478d-b796-6130d6f32ff8/12g_on2014_mastcamintovalley_live-1.jpg)
Under an exploration program begun in 1993, NASA has sent nine spacecraft to Mars, four to study the planet from orbit and five to land on its surface. Although the program has had its share of failures—two other spacecraft were launched and lost—it has also had spectacular successes. Mars Pathfinder, which landed in 1997, was aptly named. Spirit, Opportunity, and the Mars Science Laboratory Curiosity have all followed. Author Rob Manning, who has worked at the Jet Propulsion Laboratory since 1980, was chief engineer for Pathfinder and Curiosity. The following is excerpted from his recent book (with William L. Simon): Mars Rover Curiosity: An Inside Account from Curiosity’s Chief Engineer. —THE EDITORS
In 2004, the White House announced a far-reaching, long-term vision for space exploration that among other things called for a “human and robotic program to explore the solar system and beyond.” The concept included preparations for the human exploration of Mars. At the time, many people were saying that they already knew how to land humans on Mars. You might think that as the guy who had landed more stuff on Mars than anyone else on the planet, I would know what it would take to land anything there. The truth was that I knew about landing small things, things the size of a dining room table. I knew little about landing anything big enough to carry people.
In response to the White House announcement, NASA began looking for people to lead a series of 15 “capability” roadmap teams—formed around such topics as in-space transportation, human health and support systems, and scientific sensors and other measuring equipment—to help set long-term directions for the agency’s focus in the coming decades. One of the teams was to explore “human planetary landing systems,” and not long after the announcement, I was named to head that panel.
I started by lining up two co-chairs. The first was Claude Graves, who had been a leader in developing the entry systems for the return of Apollo’s astronauts from the moon to Earth. (His death in 2006 was a huge loss to the space program.) My other choice was Harrison Schmitt, known as “Jack.” He had walked on and driven around the moon as part of the Apollo 17 crew, the last Apollo moon mission. He held a doctorate in geology from Harvard University and had also served in Congress as a senator from New Mexico.
For the rest of the panel, the group I rounded up included some core space shuttle specialists in entry, descent, and landing; shuttle astronauts, and people who had built Mars landers, including Viking, the first one to land on the planet, in 1976. I also brought in a few key thinkers from industry and academia, including an old friend, Bobby Braun, a Georgia Tech professor who had helped bring entry-system aerodynamics engineering back from the edge of extinction. Others included Marshall Space Flight Center’s Michelle Monk and old hands like Dick Powell of NASA’s Langley center in Virginia, who had worked on early entry, descent, and landing—EDL—concepts for Mars and would help make sure that our team’s work was solid.
In all, I put together 50 EDL people from around the country. This would be the first time in NASA history that EDL people who built “the little ones” like Pathfinder, Spirit, and Opportunity would be formally putting their heads together with people who built “the big ones”— the spaceships that had carried humans to the moon and back, as well as the shuttle.
In December 2004 , the panel gathered for its first meeting: a day-and-a-half session at the California Institute of Technology, not far from JPL. Human missions to Mars would need to land many tons of gear in order to provide for a few astronauts to survive on the planet. In fact, given the struggle our Mars Science Laboratory team was having with a one-ton rover, I had trouble imagining how it would ever be possible to put on Mars the kind of supplies that would be required.
I am often asked why landing on Mars is so much harder than landing on the moon or on Earth. To land on the moon, the astronauts entered lunar orbit and fired retro-rockets aimed more or less opposite to their direction of travel. As their spacecraft slowed, it descended toward the surface. The landing isn’t trivial, but it’s reasonably straightforward. To bring a lander back to Earth, retro-rockets aren’t needed, because Earth has an atmosphere. Most Earth landers can eliminate more than 99 percent of the speed of orbit simply by slowing down with a heat shield. For the last one percent, we can use parachutes (as did Soyuz) or wings (as did the space shuttle).
Mars is like neither the moon nor Earth, but is annoyingly in between. It has too much atmosphere to land as we do on the moon and not enough to land as we do on Earth. The thickness of the Martian atmosphere at the surface is similar to what a mountaineer on Earth would feel if standing on top of a mountain 130,000 feet high—four and a half times higher than Mount Everest. At that altitude, the space shuttle is still screaming along at over 4,000 mph. How do you slow down quickly enough that by the time you reach the ground you’re not still going over 1,000 mph?
That’s the problem we face when designing our Mars landers. It’s the reason our machines are such Rube Goldberg-esque contraptions and why the seven minutes of entry, descent, and landing are so terrifying. We have to combine all of the tricks we use to land on Earth (heat shields, parachutes) with the techniques we use to land on the moon (retro-rockets, airbags), among many others. For the Mars Science Laboratory (later renamed Curiosity), we had decided to combine two systems, a precision-controlled propulsion system that had been pioneered on Viking and another system designed for Mars Pathfinder.
On Pathfinder, instead of falling away from the entry capsule and landing on legs, the lander was lowered on a rope, and hung under the parachute while falling through the thin Martian atmosphere at more than 150 mph. We kept the lander suspended under the parachute until the last possible second. At about 500 feet above the ground airbags were inflated and three big solid rockets fired for a couple of seconds. The lander came to almost a dead stop some 50 feet up. From there it fell free and bounced over the terrain for the next minute or so until coming to rest.
If a Pathfinder-like lander or rover had more precise control of its rockets, as the Viking spacecraft did, this propulsion system as part of an “entry and descent stage” might be able to lower the lander closer to the ground than Pathfinder’s and slow its descent enough to make a supersoft touchdown. No airbags would be needed. The design was a rover-on-a-rope concept, made more practical by Miguel San Martin, the best guidance-and-control engineer and architect at JPL.
In the same year that I convened the panel studying human landings on Mars, another group evaluated this landing system for MSL. The evaluators included an astronaut and a helicopter pilot as well as experts in mission assurance, propulsion, radar, reliability, systems, guidance, multi-body dynamics, and kinematics. The helicopter pilot pointed out that experienced heavy-lift helo pilots can control both the speed and the position of their suspended loads with exquisite precision. This was a man who had extensive experience in one of the early heavy-lift helicopters, the Sikorsky Skycrane. Afterward we started to call our landing approach the “skycrane maneuver.”
One of the biggest challenges we faced had been how the EDL software could determine when the rover had settled onto the Martian surface. On some of the prior legged-lander Mars missions, each of the three legs had a sensor that signaled the lander’s computer that it had touched down. As soon as two of the legs were down, the computer would turn off the engines. This was a crucial element; if the engines were not turned off quickly enough, the rockets could force the lander to bounce and roll over.
But how would the computer know when our rover had landed? Would we need to add complex touchdown sensors in each of the rover’s wheels?
It was Miguel San Martin who stumbled on the answer. Mig, always curious, had been playing around with some of his computer simulations. He noticed that the throttle setting dropped by half when the rover made contact with the ground. He realized that the rover weighs about as much as the descent stage does at this point—so an instant after touchdown, the thrusters would have only half as much lifting to do. He had stumbled onto the solution.
Imagine a helicopter pilot lowering a heavy container to the ground. In flight, he needs to keep a lot of power to the main rotor in order to counteract the downward pull of the container. As he descends gradually toward the delivery spot, he’s monitoring carefully; he knows that as soon as the container is on the ground, this extra power will cause the helicopter to start rising, so as soon as it begins rising, he will quickly roll off the throttle. The start upward is all the confirmation he needs that his container had landed.
What Miguel realized was that the moment of needing less thrust was the moment that the rover had touched down. When Miguel’s trick had been pointed out to us, we could hardly believe it. The issue we had been struggling with for months had a quite simple solution that required no special touchdown detection sensors at all! The rover software had to do just two things for touchdown: maintain the slow and steady descent rate during the skycrane phase, continuing that slow rate until the ropes started to become slack, and then monitor the engine throttle setting to be sure that once the power had been cut, the situation remained stable, confirming that the rover was firmly on the ground. That’s the system that made history by safely landing our one-ton rover Curiosity on Mars.
Landing humans on Mars would be a much tougher problem. To kick off our panel’s first session, after everyone had introduced himself or herself and provided a brief background, I asked them all to share what I called their “care-abouts.” What did they think the major issues were?
Kent Joosten, a brilliant design architect of human Mars missions, explained NASA’s “short- and long-stay” mission designs. Long stays would require the astronauts to be on Mars for 545 days (530 Martian days, or “sols”) and away from Earth for two and a half years. Short stays would require “only” 40 days on the surface and a little more than one and a half years away from home. In either case, the stuff that needed to be landed for the astronauts would be massive.
From Kent, we heard the description of a whole stocked-up camp that would be placed robotically prior to the astronauts’ arrival. To save weight, water would be extracted from the Martian air, and the oxidizer for the fuel needed to take off for the journey back to Earth would be manufactured on Mars. Radioactive power plants and solar arrays would be brought in by spacecraft, ready to be set up by humans. Even the crew’s Mars ascent vehicle for the return home would be positioned in advance, fueled and ready to go.
Jack Schmitt next took the floor and explained what a Mars mission would look like from an astronaut’s point of view. Being in space was lonely enough, he said. Astronauts would need people to talk to, people in mission control to help work them through the tough spots. But mission control in Houston is between 11 and 22 light-minutes away, depending on where Mars and Earth are located in their orbits. You could talk for 10 or 20 minutes before the first word you uttered ever arrived at an Earthling’s ears.
An unexpected issue surfaced when Jack commented, “To do this landing safely, of course, we need the ability for the astronauts at any time to hit the abort button, wave off the landing approach, and go back into outer space.”
Bobby, Dick, and I glanced at one another, appalled, and we all looked at Jack with stunned expressions that translated as, “What? Are you kidding? How the heck could we do that?”
With Mars landers, aborting raises a much bigger problem than landing. All of our Mars landers and rovers, as they approached the planet, shed an assemblage of intricate hardware and components, leaving a trail of debris on the way to the surface. We assumed that a human mission would do the same. How could it do all of those transformations and undressings, while still being able to turn around to fly back up into outer space in an emergency?
When we explained that the Mars entry was more like landing on Earth than landing on the moon, the astronauts were quite surprised. We gave them an explanation that went something like this: “Imagine you’re going at Mach 15 surrounded by a bubble of hot gas as you plunge through the Martian upper atmosphere. You’re decelerating at up to 6 Gs. If you wanted to change your mind and head back to space, how would you do that? You’re going so fast that there’s no way to undress yourself from the heat shield and turn your craft into a rocket. Worse, you’re headed in the wrong direction going extremely fast. The amount of fuel you would have to have at that point to get back into space would be enormous.”
The robotic EDLers in the crowd were thrown for another loop when shuttle astronaut Commander Barry (Butch) Wilmore argued the need for the astronauts to be able to take over control during landing on Mars, just as Neil Armstrong had to do during the first lunar landing. I know that if I were an astronaut, I’d want to be able to control my spaceship. But those of us who design rovers to land on Mars also know that those landings are nothing like landing on the moon or landing the space shuttle back on Earth. The Martian atmosphere is shallow; its top is so close to the surface of the planet that events during landing unfold at lightning speed.
We’re talking about an astronaut who has been traveling through deep space in zero G for well over a year and is now undergoing extreme entry deceleration. Silicon chips running at 120 million operations a second can handle it, but I wouldn’t expect any human—not even a highly trained, exceptionally fit astronaut—to be able to do the same. The entire sequence of events for entry, descent, and landing would almost certainly need to be automated.
Over the course of three months, we gathered for two additional multi-day workshops. Gradually we started to home in on a few key observations and details about putting human-scale landers on Mars. What became clear to me was that in the 50 years of its existence, NASA had never really had the focus, resources, or real Mars landing experiences to study these problems clearly.
One issue that was primary for me is representative of the back-and-forth that the panel went through on many topics: The items astronauts would need for a longer stay would fill pages and include food, fuel, oxygen, energy, breathable air, some type of living quarters, roving equipment, and a return vehicle. Even if we counted on pre-supply missions, they would probably require a spacecraft of 30 to 70 metric tons. How could we possibly bring that much stuff to a stop at a precise location on Mars?
When the conversation turned to decelerating from supersonic speeds, the first item addressed was parachutes. In the group were two of the nation’s leading experts in parachute design. Juan Cruz, from the NASA Langley Space Center in Virginia, is a part-time professor at Georgia Tech and has a reputation as NASA’s best expert for supersonic parachutes and supersonic deceleration. He and I knew each other well, since he had worked on the chutes for Spirit and Opportunity and was deeply involved in the design analysis for the MSL parachute. We also had on the panel a longtime builder of Mars parachutes. Al Witkowski, of Pioneer Airspace, is one of the world’s most prolific designers of aerospace chutes.
Juan explained the fundamentals and design limitation of large supersonic chutes. He reminded us that the largest ever tested supersonically was about 85 feet in diameter. But the people who had been asked to do human Mars EDL simulations years earlier reminded us that they had done computer studies of simulated but much larger parachutes that would allow a human-scale lander to slow from somewhere just above Mach 3 down to less than Mach 1 over a few tens of seconds, but with a rather large back-breaking jerk as the parachute inflated.
Al and Juan just laughed. Juan told them, “We can’t scale parachutes up to the size of the Rose Bowl! Not without understanding the physics of parachute deployment and inflation of something that size. We can’t even say that the parachute will open in time before the lander hits the ground.”
I asked Juan, “Are you telling us we don’t have any parachute solution for going from supersonic speed down to subsonic speed for a large-scale lander?”
“That’s right,” he said.
The room went silent.
We created a subgroup to brainstorm various technology options to address the issue of slowing. Its members came back with an alphabet soup of possible technologies, but by then it was beginning to sink in: At this stage, none of the experts was able to offer a single effective way of slowing down.
We spent the last day brainstorming a schedule or timeline that we would need to solve the EDL problem and get it to the point that it would be usable for landing humans on Mars. We figured that, like the Apollo project, one or more Earth flight tests of bits and pieces of the full-scale EDL design would be required.
Although I was not a big fan of the idea, the team also assumed that we would at some point land at least one smaller robotic Mars mission to test some of the new technologies. When you stacked all of these up and counted forward, we calculated that the final EDL design concept would have to be ready by 2015. On that timetable, the first human footprints would not appear on the Martian soil until about 2032, and that would be if the project were funded soon.
Budgetary worries aside, in these fascinating workshops spread over six months, my human planetary roadmap team had created guidelines for dealing with some of the more challenging technical problems we’ll face in the effort to land humans on Mars. As an outcome of our efforts, some of the needed technologies are actually being developed right now.
Excerpted From
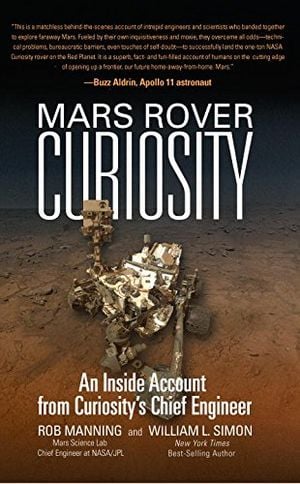
Mars Rover Curiosity: An Inside Account from Curiosity's Chief Engineer